Introduction
What flow rate to use with a patient hood is a question with many implications. Hood flow rate primarily affects patient inspired CO2 levels, humidity level, hood noise level and oxygen consumption. It can also have substantial effects on breathing pressures and patient comfort. For many years, “standard practice” for management of patient hoods had been to supply them with a steady flow of about 28 to 30 actual liters per minute (alpm, actual volumetric liters at chamber conditions) regardless of the size of the patient. However, the scientific basis for that practice is obscure. This paper presents an analysis of the flow dynamics of patient hoods and the associated implications regarding hood flow rate selection.
Methods
The model
The flow dynamics of patient hoods closely resemble those of neckdam style air diving helmets. The performance aspects of such helmets have been extensively studied by the navies of the world and are well known.1 Using the principles established for air diving helmets a mathematical model of a hood was created as shown in Figure 1.
The CO2 results predicted by the model used for this analysis were tested according to published procedures2 for open circuit air diving helmets
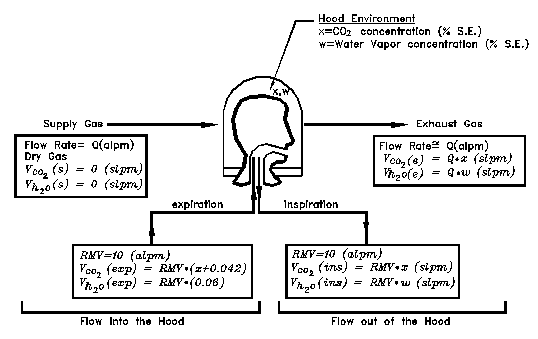
Figure 1: Hood Ventilation Model
Key assumptions and conventions are:
• The gas in the hood is assumed to be “perfectly mixed”. Transient affects are ignored and there is assumed to be no preferential exhausting of the patient’s expired gases and no pooling of expired gases.
• The patient’s inspiratory and expiratory flows, which are highly pulsatile, are converted into their steady-state equivalents. This assumption has been found to be reasonably accurate for this type of device2, and it enormously simplifies the calculations.
• At the activity levels of interest, Respiratory Minute Ventilation (RMV) in actual liters per minute (alpm) is taken as 20 times oxygen consumption in standard liters per minute (oxygen consumption equals 5% of RMV)1 . At higher activity levels RMV increases to 25 times oxygen consumption. (oxygen consumption = 4% of RMV)1
• Carbon dioxide production is taken as 84% of oxygen consumption (respiratory quotient = 0.84).
• The patient’s expired gas is taken as saturated at body temperature. This produces a water vapor content of 6% surface equivalent in the patient’s expired gas.
• The supply gas is assumed to be dry, 100% oxygen.
• Concentrations are expressed in terms of % surface equivalent. Metabolic flow rates (oxygen consumption and CO2 production) are expressed in terms of standard liters per minute (slpm). Hood flow rate is expressed in terms of actual liters per minute (alpm). This combination of units removes chamber pressure from the calculations.
Flow through the hood expressed in terms of true volume at chamber conditions is the “carrier” parameter to which all other parameters are related. CO2 production expressed in terms of standard liters per minute (which assumes a pressure of 1 ATA) when divided by true volumetric flow rate (alpm) converts directly to CO2 concentration per unit volume, which is a definition of partial pressure. When this concentration is expressed in terms of percent surface equivalent (% S.E.), no conversion constants are required and the equations simplify. For example, a CO2 flow component of 2 slpm in an overall flow of 50 alpm produces a CO2 concentration of 4% S.E. regardless of the value of the local total pressure.
Patient Activity Levels and Associated Oxygen Consumption Rates
Patient activity levels were looked at from two viewpoints. The first was air conditioning technology3 which focuses more on the total heat produced by a person. The second viewpoint was physiology4 which focuses more on tissue oxygen consumption. When both approaches are compared for a 160 lb (72.6 Kg) male patient and normalized on total oxygen consumption at the Seated Quietly condition, the results are as shown below.
a. Air conditioning terminology3: 1 Met = an energy production of 58.2 watts per square meter of body surface area. In this system an average man is assumed to have a weight of 154 lb (70 Kg) and body surface area of 1.8 m2. Such a person at 1 Met has an oxygen consumption of 0.308 slpm (0.32 slpm for the 160 lb person assumed.)
b. Physiology terminology4: 1 METS = 3.5 ml oxygen consumption per kg per minute assuming resting conditions.
Two activity levels were examined, seated quietly and standing quietly. It is reasonable to assume that the metabolic demand on most patients, even with a reasonable degree of anxiety, will fall somewhere between these two conditions with a tendency to favor the seated quietly condition.
The physiology system, METS, relates oxygen consumption directly to body weight. Accordingly, the results of this analysis are presented on the assumption that a patient’s oxygen consumption, and therefore his RMV, CO2 production and water vapor production are directly related to his body weight. This is a reasonable assumption for most patients.
However, for extreme cases or in cases where oxygen supplies are limited, some corrections based on air conditioning data3 may be useful as follows;
a. In the air conditioning system, oxygen consumption is related directly to unit body surface area and then indirectly to weight according to
Ad = 0.202 w0.425 h0.725
where Ad is total body surface area in square meters, w is both weight in kg and h is height in meters. This suggests that for very obese patients, setting hood flow rates solely on the basis of body weight will result in flow rates that are substantially too high. For such patients, in may be more appropriate to use an “effective weight” computed according to
Weff = Wn * (Wa / Wn)0.425
where Weff = effective weight for use in the charts, Wa = actual weight and Wn = normal weight for the patient’s height
b. Women and children are estimated to expend about 10% more energy per unit body weight. Therefore, Weff = actual body weight plus 10% will be more appropriate.
c. Energy expenditures decreases about 2% per decade after age 20. Therefore for very elderly patients, Weff can be reduced accordingly.
Target Conditions
Carbon Dioxide. A CO2 concentration of 2.0% surface equivalent (15.2 mmHg partial pressure has long been regarded as a safe inspiratory CO2 level for divers who must retain intellectual acuity despite long exposures, cold and hard work1. That level normally produces no increase in minute ventilation and can be tolerated for long period of time. It is taken as the target upper limit for permissible hood CO2 levels. However, it is not a “hard” limit. In normal healthy individuals, CO2 levels of up 3.0% surface equivalent can usually be tolerated for short periods of time without significant discomfort and for extended periods of time without harm.6
Water vapor (humidity). Very low levels of humidity in a hood can become uncomfortable to the patient and can lead to the accumulation of static charge. (In extreme cases patient’s hair has been known to stand up.) Humidity levels in the range of 50 to 70% are considered comfortable for hyperbaric chambers5. At an environmental temperature of 70oF (21oC), the maximum partial pressure of water vapor that can be present without condensate forming is 2.4% surface equivalent (18.8 mmHg partial pressure). Therefore, the target water vapor level is taken as less than 2.4% S.E. (100% relative humidity, R.H., at a hood temperature of 70oF) and more than 1.2% S.E. (50% relative humidity).
RESULTS
Hood Flow Rates Required to Produce the Target Conditions
Application of the principal of conservation of mass (total inflows must equal total outflows) to the model shown in Figure 1 results in the following equations for hood CO2 and water vapor concentrations:
This suggests that the preferred range for Q is greater than 2.1 times RMV and less than 4.0 times RMV. However, as previously discussed, RMV correlates with oxygen consumption which further correlates with patient body weight and activity level. Consequently, for specified patient activity levels, the preferred range of hood flow rates, Q, can be correlated directly with patient body weight. The results of this correlation are shown in Figures 2 and 3.
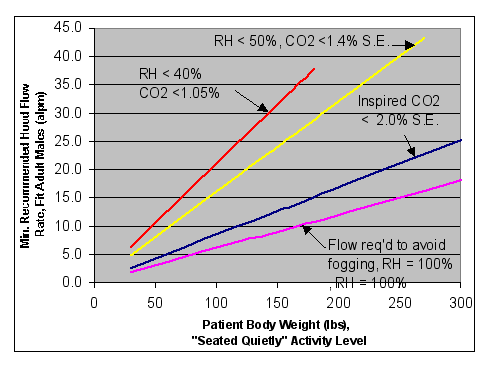
Figure 2, Effect of Hood Flow Rates on Inspired CO2 and Humidity Levels
for Patients “Seated Quietly”
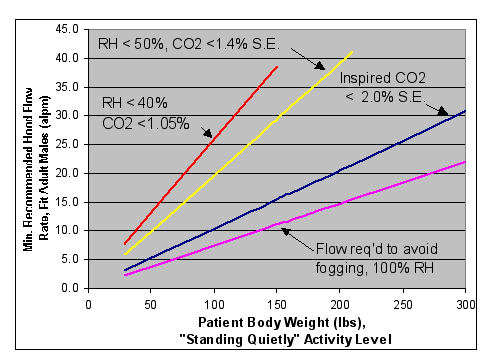
Figure 3, Effect of Hood Flow Rates on Inspired CO2 and Humidity Levels
for Patients “Standing Quietly”
Practical Implications
From Figures 2 and 3 it is clear that the issue of hood flow rate is not a “one size fits all situation” as has historically been common practice. Large patients may require flow rates well above the customary 1 acfm (28.3 alpm) value to be comfortable. Smaller patients can safely use lower flow rates and may require them if the supply gas is not humidified. This is especially important in the case of children.
Since the hood flow rates that result in 100% humidity are below the rates required for the target 2.0% S.E. inspired humidity level, any sign of fogging in the hood means the hood supply flow is too low and should be increased.
The 100% R.H line corresponds to an inspired CO2 level of 2.8% S.E. which is above the target level, but still normally tolerable. Consequently, for most patients inspired CO2 levels are not a significant concern so long as the hood does not fog. However, patients with a CO2 retentive condition or other condition that may make them unusually sensitive to inspired CO2 levels may require higher minimum hood flow rates.
The 2.0% S.E. CO2 line corresponds to a humidity level of 80%. Most patients will find this humidity level uncomfortable. The comfort zone for most patients can be expected to be from slightly above the 2.0% S.E. CO2 line to slightly above the 50% RH line.
With proper attention to the relationship between hood flow rate and patient body weight, the need to humidify the hood supply gas can be avoided. For children and very small adults, existing hood flow controls may not work well at flows low enough to maintain at least 40% humidity. In such cases humidification of the supply gas may be necessary. .
Figure 2 shows that a hood flow of 20 alpm should be satisfactory for most patients. Current practice is to supply hoods at approximately 1 acfm (28.3 alpm). If an average hood flow rate across all patients of, for example, 22 alpm could be achieved, the savings in facility oxygen consumption would be about 22%. This can be significant in locations where oxygen is expensive or hard to obtain.
To effectively use the information provided in Figures 2 and 3, the hoods must be fitted with flowmeters that read in meaningful units. Most flowmeters in current use are of the variable area type and are fitted with scales that are accurate only when the flowmeter is used in a one ATA environment. The general calibration equation for variable area flowmeters used at other than calibration conditions is complex. However, for the typical case of flowmeters calibrated at one atmosphere and used for the same gas in a hyperbaric chamber where the pressure inside the flowmeter equals chamber pressure, the calibration equation simplifies substantially and results in the equations shown in Fig. 4.
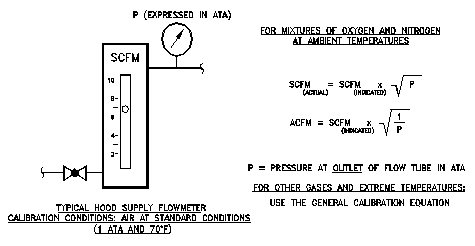
Figure 4 How To Read The Most Commonly Used Type of Hood Flowmeters.
For the above equations to be valid, P must be the pressure in the flowmeter exhaust port expressed in atmospheres absolute (ATA). There cannot be any flow control devices between the flowmeter and the patient hood.
SUMMARY
The current practice of supplying hoods at a rate of nominally 1 acfm (28.3 liters per minute) is satisfactory for most adult patients. However, very large patients may require more flow, very small patients may require less flow especially if the gas is not humidified and most patients should be comfortable with up to 20% less than the customary 1 acfm.
Understanding what is happening in hoods makes it possible to maximize patient comfort and minimize oxygen consumption. Minimizing oxygen consumption can be vitally important in locations where oxygen is expensive or difficult to obtain such as nearly all island communities.
REFERENCES:
- Morrison, J.B. and Reimers, S.D., “Design Principles of Underwater Breathing Apparatus,” Chapter 3, Physiology and Medicine of Diving, 3rd Edition, Editors P.B. Bennett and D.H. Elliot, Brailliere Tindall Press., London, 1982
- Reimers, S.D., “Testing Procedures for Open Circuit Air Diving Helmets And Semi-Closed Circuit Mixed Gas Diving Helmets,” U.S. Navy Experimental Diving Unit Report 20-74, .December 1973
- ASHRAE Handbook, 1977 Fundamentals, American Society of Heating, Refrigerating and Air-Conditioning Engineers, New York, New York, 1978
- Guyton, A. C. and Hall, J.D., Textbook of Medical Physiology, 10th Ed., Philadelphia: W. B. Saunders Company, 2000
- Chapter 19, “Hyperbaric Facilities”, NFPA 99 Health Care Facilities, 1996 Edition, National Fire Protection Association, Quincy, MA 1996
- U. S. Navy Diving Gas Manual, 2nd Edition, NAVSHIPS 0994-003-7010, U.S. Navy Supervisor of Diving, Washington, DC 1971